Feedback and Fidelity – by Norman Koren
Negative feedback can be a wonderful thing when done well,
but it’s often done badly.
Here’s how to use it properly.
Table of contents
Part 1 – Negative feedback concepts and preamplifiers
- Introduction
- Computer modeling
- Models and measurements
- The trouble with feedback Instability
- Output transformers
- RF interference
Part 2 – Feedback in power amplifiers
- Clipping
- Harmonic distortion scam Better measurements
- Feedback forever?
- Feedback without fear
- Output connections
Negative feedback concepts and preamplifiers
I’ve seen negative feedback attacked, reviled, denounced, and defamed for so long I can no longer stand idly by. Feedback may be as stylish as a ‘63 Dodge Dart, but it’s a dear friend who’s brought beauty and joy to my life. It may not be a saint, but we don’t expect Marilyn Monroe to be Mother Theresa, do we? It’s just misunderstood— often as much by its supporters as by its detractors. In the ten years I’ve been designing and building amplifiers I’ve learned how to bring out its best while respecting its limitations. The time has come to share that knowledge.
In his January 1998 Stereophile article “A Future Without Feedback ,” Martin Colloms asserts that “measurements do not fully describe sound quality,” and goes on to suggest that “corrective feedback is fundamentally unmusical.” He poses the question, “has anyone explored the implications of negative feedback for reproduced sound quality in the absolute sense?” What follows is the results of such an exploration. We shall describe new measurements that provide improved insight into the origins of audio quality, and we shall use these measurements to determine when and where to apply feedback to best advantage. Although we will use vacuum tube circuits as examples, all observations apply equally well to solid-state. We shall also revisit traditional measurements. One of them— harmonic distortion at rated power output— has an unexpected correlation with sound quality.
Sometimes it seems that so much has been written about feedback’s harmful effects that it’s easy to forget its benefits. Why do engineers love it? Improved frequency response, reduced harmonic distortion, better gain control, increased input impedance (in many circuits), and decreased output impedance (in circuits with voltage feedback). So why do audiophiles hate it? Harsh, gritty, grainy, glaring sound. Reason enough.
Now for the BIG question: Are these qualities intrinsic to negative feedback or do they arise from its improper application? From my experience it’s mostly the latter. Mostly. There are a few places where feedback runs into unavoidable problems, and it shouldn’t be a big surprise that one of them is the single-ended vacuum tube power amplifier. How can we know this? We have an instrument for looking deep into the heart of amplifiers— an instrument that may be within your reach this very moment.
The idea of using the computer— the ultimate digital machine— to design old-fashioned vacuum tube circuits may seem more than a little incongruous. So it may be, but in the computer we have powerful tool that wasn’t available in the glory days of Dynaco, McIntosh, and Marantz; an affordable tool for anyone interested in high-end audio, and usable by anyone with modest engineering skill. More precisely, our tool is a computer program called SPICE— acronym for Simulation Program with Integrated Circuit Emphasis, originally developed at the University of California Berkeley.
SPICE is widely used in industry to prove integrated circuit designs before they are cast in silicon, where fixing errors is far more costly than in concrete. There are several commercial versions of SPICE, all of which start with the Berkeley algorithms and add user-friendly front-ends and outputs. Probably the best-known of them is PSpice from Cadence Design Systems (https://www.orcad.com/Product/Analog/analog.asp). A free evaluation version that can simulate up to fifty parts is available on CD ROM or can be downloaded from the web. Fifty parts won’t get you far with semiconductors, but it’s sufficient for the design of surprisingly sophisticated vacuum tube circuits. Full versions of PSpice are very expensive. Another excellent program is Electronics Workbench from Interactive Image Technologies (1-800-263-5552; https://www.interactiv.com), which comes in a $299 package (sometimes on sale) that can do some serious simulation. Each of these programs has its learning curve, and since I’ve taken the trouble to learn PSpice, I’m stuck with it. I love it.
I’m not the only one who values SPICE. When I escaped from Silicon Valley in 1985, I had a neighbor who was developing a version (HSpice) in his garage. While I was tinkering with tubes, he was quietly taking over the market for large-scale integrated circuit simulation. In August 1997 I read that he had sold his company— Meta Software— for one hundred and sixty million dollars. (That was before the dot com boom, when hardware still got some respect.) Can there be a lesson here? I suppose we tube lovers must be content to receive our reward in heavenly sound. None of us will become another Bill Gates. Besides, the only reproduced sound he ever hears is digital.
Many readers may wonder how well digital simulation can unlock the secrets of analog electronics. In my experience it can do so astonishingly well. I never cease to be amazed by how closely measurements match SPICE simulations.
No matter how strongly you believe that measurements don’t or can’t correlate with sound quality, you must agree that electrical signals inside circuits obey the laws of physics. If a computer program has accurate enough device models, it will simulate the signals with precision. If you examine the details of those signals with enough care, you may begin to find patterns that shed light on sound quality.
What is wrong with conventional measurements? Two things. The first is that most of them are made in frequency domain. The real world happens in time domain. Frequency domain measurements are derived from a mathematical construct called the Fourier transform, which is defined for linear systems. When a system becomes seriously nonlinear— as an amplifier does when it saturates— frequency domain measurements their meaning. Time domain measurements, such as pictures of clipped sine waves, are needed to tell the real story. The one time domain measurement frequently seen in equipment reviews is the 10kHz square wave. This measurement is usually made with a small signal— far from saturation— and provides the same information as the frequency response curve. SPICE produces output in both time and frequency domain.
The second problem is that conventional measurements are taken only at an amplifier’s external connections: A signal is fed into the input terminals and measured at the output terminals. What happens inside the circuit can make the difference between sonic mediocrity and distinction. With SPICE, you can probe deep inside of circuits. I’ve made measurements that would be difficult, expensive, and time-consuming with hardware instruments; measurements rarely if ever seen in equipment reviews; measurements that correlate much more closely with sound quality than such old standbys as harmonic distortion and frequency response. We’ve known for a long time they didn’t hold the secrets.
A program’s performance is only as good as its models— sets of equations that simulate device behavior— and SPICE does not have built-in models for vacuum tubes. External models must be added. For many years tubes were modeled by the Langmuir-Childs law
A new set of models, accurate enough to match experimental tube behavior in all critical regions, has recently been published [3] and applied to the design of a modified Dynaco PAS preamplifier [4] with stunning sonic results. We shall use the old and new PAS line amplifiers (figures 1 and 2) as examples of problems and solutions related to feedback.
To you non-technical readers, I offer an apology. Feedback cannot be discussed intelligently without getting somewhat technical. I shall try to keep this exposition as readable as possible— There will be no heavy formulas, and you may safely skip over circuit descriptions and references to resistors and capacitors.
Before we proceed, a few definitions are needed: There are two types of negative feedback, or degenerative feedback as it is sometimes called: local and global. Local feedback is connected within or around a single gain stage; global feedback is connected around several gain stages, usually from the amplifier’s output to its input. Local feedback is generally regarded as benign, and with this view I concur. The amount of feedback, expressed in decibels (dB), is the ratio of the gains without and with feedback (the open and closed-loop gains): 6dB is a factor of 2 in voltage (4 in power); 20dB is a factor of 10 in voltage (100 in power), etc.
Despite its advantages, negative feedback can degrade amplifier sound quality in three ways: First, it can lead to instabilities that appear as response peaks or even oscillations at an amplifier’s frequency extremes. Second, it can increase susceptibility to RF interference. Third, it makes clipping more abrupt: This rarely affects preamplifiers, which have plenty of headroom, but it is always a concern in power amplifiers. With proper design, the first two problems can be eliminated and the third can be controlled. We shall examine each of them closely with the help of SPICE.
Negative feedback operates by subtracting a portion of an amplifier’s output signal from its input. This is quite straightforward for the middle frequencies where an amplifier’s open-loop gain is relatively flat. Bode’s theorem tells us that there is very little phase shift in this region. But things can get ugly at the frequency extremes. Every RC (resistor-capacitor) network that contributes to an amplifier’s rolloff adds up to 6dB per octave to the rolloff and up to 90 degrees to the phase shift. Not all of these RC networks are obvious in the schematic: Many involve stray capacitances within the tubes. If the total phase shift exceeds 180 degrees at any frequency where the loop gain (A/G-1, where A is the open-loop gain and G is the closed-loop gain) is greater than one, the amplifier will oscillate [5]. If it merely approaches 180 degrees, a peak will appear in the frequency response curve that corresponds to ringing in the time-domain. This will definitely degrade sound quality.
To make matters worse, capacitance in shunt with the load increases the phase shift. Interconnect cables have around 20pF per foot, and electrostatic loudspeakers are nothing more than big honking capacitors– as large as 2 microfarads– that know how to move. So load capacitance is always present. An amplifier that performs nicely with a purely resistive load (widely used in equipment reviews) may misbehave in the real world. Amplifiers have a property called phase margin— the difference between the maximum phase shift and 180 degrees— that indicates how well they can tolerate capacitive loads. A qualitative estimate of phase margin may be obtained by measuring the response with capacitance in shunt with the load. This is particularly easy with SPICE.
To keep phase shift under control, a single RC network must dominate the rolloff. For global feedback loops, this usually involves adding a capacitor to the circuit. This is accomplished in the original PAS line amplifier (fig. 1) with 33pF capacitor CLFB connected in parallel with feedback resistor RLFB, and in the new design (fig. 2), with 7pF capacitor C3M in the input circuit. Although these capacitors reduce the high frequency cutoff (-3dB point), it is still around 100kHz in both circuits— well beyond the limits of human hearing. Most well-designed preamplifiers achieve good frequency response and stability at the same time— but this is not always the case for power amplifiers with output transformers.
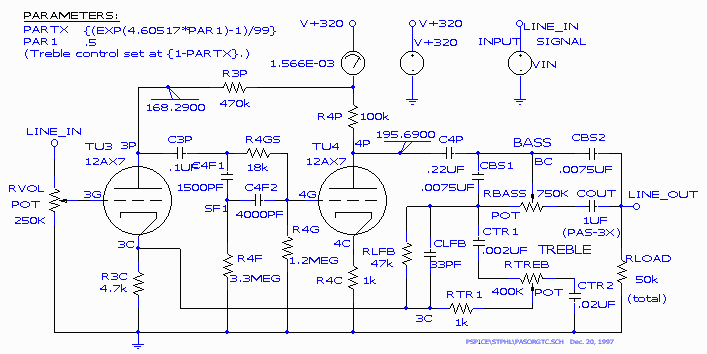
Figure 1. The original PAS line amplifier
The line amplifier of fig. 2 differs in just one respect from the previously published version [4]: the addition of 150 ohm resistor ROUT in series with the output. I had always objected to such a resistor because it increases the output impedance, worsening the high frequency rolloff in the presence of shunt capacitance from cables. But with negative feedback, phase shift due to shunt capacitance boosts the high frequency response up to the resonant peak, just over 100kHz. In extreme cases, this can even result in oscillation. Increasing the compensation capacitor fixes this problem at the expense of high frequency rolloff for short cables with low capacitance. ROUT solves the problem by isolating the amplifier from the load capacitance. If the correct value is chosen (easily done by trial-and-error with SPICE), the high frequency loss due to the increased output impedance will balance the boost due to feedback, making the response below the resonant peak nearly independent of the load capacitance— even for cables as long as 100 meters. This neat little trick cannot be accomplished without negative feedback.
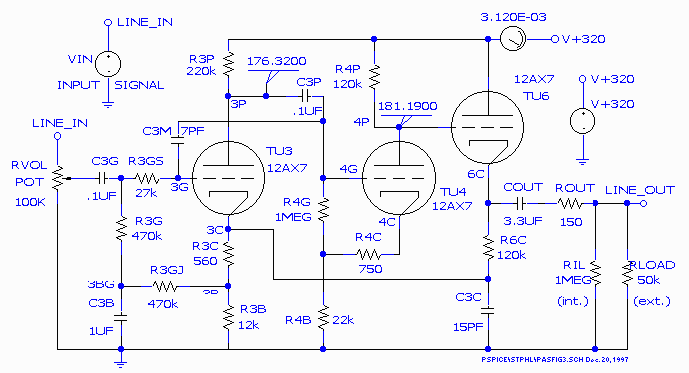
Figure 2. The modified PAS line amplifier
If we observe the response of the original PAS line amplifier (fig. 3; simulated with Evaluation PSpice 6.3, which tolerates more components than the newer evaluation versions), we find that the output signal is fairly flat to below 1Hz, but the signal at the plate of the first gain stage plate (node 3P) has a huge resonant peak at 0.4Hz— 35dB above the mid-frequency level. This peak is present because there is no feedback at dc: It is blocked from reaching the output terminal by coupling capacitor C4P.
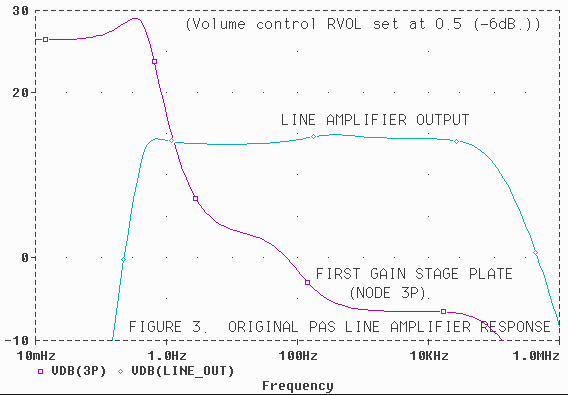
Fig. 3. Original PAS line amplifier frequency response (first plate 3P and output LINE_OUT)
Now you might ask what effect a resonant peak far below the limits of human hearing and loudspeakers— a peak that doesn’t even appear at the preamplifier output— can have on sound quality. Quite a lot, it turns out. To begin with, 33 RPM turntables rotate at a frequency close to 0.4Hz. Signals from mechanical perturbations at this frequency will be greatly exaggerated inside the preamplifier. Then there is tube-generated 1/f noise, also called flicker or pink noise— noise whose power spectral density is inversely proportional to frequency (f). 1/f noise can be very significant at 0.4Hz. These two noise sources perturb the bias levels of tubes inside the preamplifier. Since the tubes aren’t perfectly linear, this leads to envelope modulation— small variations in signal amplitude at the output that muddy the sound and blur the imaging.
This problem has been solved in the modified PAS line amplifier (fig. 2) with a dc-coupled negative feedback loop: Cathode resistor R6C of the cathode-follower also functions as the feedback resistor. It took quite a bit of trial-and-error with SPICE to get the input tube operating current to the correct level (0.7mA; much higher than the original). A resonant peak (fig. 4) is still present in the new circuit, but it is now 3dB below the mid-frequency signal level— 38dB better than the original. This is a perfect example of how external measurements can be misleading: The original design has much more extended low frequency response, but the new design has cleaner bass and better overall sound.
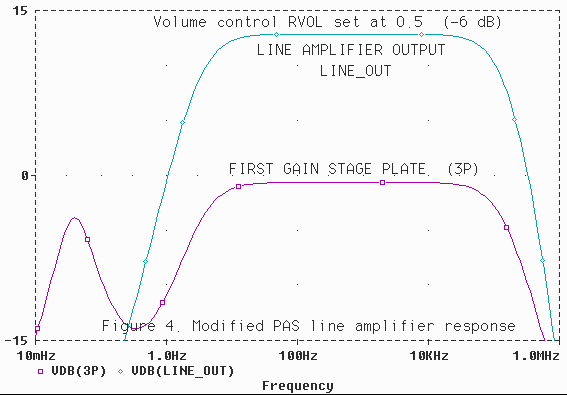
Fig. 4. Modified PAS line amplifier frequency response (first plate 3P and output LINE_OUT)
Reduced to its essentials, an output transformer is a device comprising two electrical windings— a primary with NP turns and a secondary with NS turns— coupled by a magnetic core, and used to match the high impedance of output tubes to relatively low impedance of loudspeakers. In simulations, transformers are modeled as devices that multiply the signal voltage by NS/NP, and have a small series inductance called leakage inductance (LSP), a large shunt inductance (LP), and a shunt capacitance (CIP). This terminology is from “Transformers and Tubes in Power Amplifiers” by Menno Van der Veen, available from Plitron (1-800-754-8766; https://www.plitron.com)— strongly recommended to anyone who wishes to pursue this subject further. Van der Veen defines the quality factor of an output transformer as the ratio of shunt to leakage inductance, QF = LP/LSP. QFis roughly proportional to the ratio of the upper to lower cutoff frequencies. Leakage inductance LSP arises from imperfect coupling between the primary and secondary windings, and is a strong function of the type of transformer. There are three broad categories:
- Push-pull EI transformers (named after the shape of the core): used in most of the classic designs. Typical cutoff frequencies are between 25 and 70kHz. Can be thought of as occupying the middle ground between SE and toroidal transformers.
- Single-ended (SE) transformers: must tolerate the large dc current drawn by SE triodes operating in class A. They have EI cores with air gaps to keep them from saturating. The air gap increases leakage inductance LSP. QF is lower than for the other types. Cutoff frequencies can dip into the audio range.
- Push-pull toroidal (bagel-shaped) transformers: Have the largest QF: Cutoff frequencies can exceed 200kHz. They are sensitive to dc-imbalance in push-pull circuits, but techniques are available to maintain balance.
An output transformer’s high frequency rolloff is dominated by the LC (inductor-capacitor) network consisting of LSP and CIP. This type of network has a second order rolloff— up to 12dB per octave at frequencies above cutoff, with a phase shift of up to 180 degrees. As a result of this phase shift, even a few dB of feedback can cause trouble— response peaks or oscillations with capacitive loads— unless the loop is carefully compensated. The compensation techniques are the same ones used in the line stages of figures 1 and 2. The high frequency cutoff of the RC network must be well below that of the transformer— the higher the feedback, the lower it must be. For this reason, feedback cannot be used to extend the frequency response of an amplifier with an output transformer. Sad to say, not all tube amplifiers apply feedback correctly. Frequency response peaks above 20kHz and ringing in response to 10kHz square waves have appeared in several Stereophile equipment reviews, even for some extremely expensive amplifiers. Such amplifiers can misbehave badly if there is much capacitance in the load.
Feedback is particularly touchy in single-ended circuits because the output transformer cutoff frequency is so close to the top of the audible range. A feedback loop must be compensated with extreme care: Too much compensation rolls off the highs very audibly; too little risks a response peak. Even just the right balance may have poor phase margin: It might sound wonderful with one speaker-cable combination but poor with others. This is one of two reasons that SE amplifiers are poorly suited for feedback. The other— hardened clipping— will be discussed below.
Feedback’s least-known side-effect is that it can increase an amplifier’s susceptibility to RF interference. This is so because the circuit technique most often employed to stabilize a feedback loop— a capacitor in shunt with the feedback resistor (CLFB in fig. 1)— allows RF signals picked up by the output cable to be fed back into the amplifier’s input virtually without attenuation. Cables are antennas, albeit poor ones, and vary widely in their sensitivity to RF pickup. Premium interconnect cables should be exceptionally well shielded to minimize this sensitivity. Speaker cables, which are usually unshielded, are much better antennas than interconnect cables. I am convinced that RF interference is a major culprit because every circuit change I’ve ever made to reduce the RF entering an amplifier has improved the sound— made it sweeter, smoother, cleaner, and more generally listenable. It is the apparent cause of the grittiness, graininess, harshness, and listener fatigue — what Martin Colloms calls “sand in the tweeter” — that can plague amplifiers with otherwise excellent specifications. The sonic degradation caused by RF interference is far worse than harmonic distortion.
RF interference comes from a wide variety of sources: radio, TV, digital cellular phones, microwave ovens, lamp dimmers, flourescent lights, and digital appliances such as computers and CD players. (It is particularly difficult to eliminate inside CD players, where it may be as responsible as jitter for “digititis.”) It is virtually omnipresent in urban, suburban, and all but the most remote rural areas. It varies from time-to-time and place-to-place, and may be responsible for many of the discrepancies in published amplifier reviews.
The exact mechanism by which RF interference degrades audio quality is not well-understood. The most likely cause is intermodulation distortion. Paul Miller [6] described a series of experiments in which he inserted strong RF signals modulated with random audio noise into several amplifiers and measured the resulting audio noise spectra (the result of intermodulation). He claimed to find a strong correlation with the subjective sound quality.
There is a very simple test for determining if an amplifier is overly sensitive to RF interference: Turn the volume up and listen for a pop when you turn on a nearby appliance. A well-designed amplifier will remain silent.
The RF problem was solved in the modified PAS line amplifier (fig. 2) by compensating the feedback loop with an RC network in the input stage— R3GS and C3M. Due to the Miller effect, the effective capacitance C3M is its intrinsic capacitance (7pF) multiplied by the gain of tube TU3 after feedback is applied (not its intrinsic gain). Calculating this gain can be tricky: Global feedback reduces the gain of the first stage only; all others operate at full gain. SPICE eliminates the need for tricky calculations. The correct value of C3M can be quickly determined by trial-and-error. This technique, which we shall call Miller compensation, has poorer high frequency response than the traditional technique, but the response can be extended— made as good as the traditional technique without compromising stability— by adding shunt capacitor C3C to the input stage cathode circuit. C3C also provides a bit of extra RF suppression.
The improvement brought about by this technique is illustrated in fig. 5, which shows the signal on the plate of the first gain stage (node 3P) when input signal VIN is applied to the line amplifier output terminal, LINE_OUT. In the critical region above 20kHz, the response of the modified amplifier averages 18dB below that of the original. The actual improvement is even greater because, with VIN applied to the line amplifier input, the signal at node 3P (figures 3, 4) is 6dB larger in the modified line amplifier. This is so because local current feedback decreases the gain of the second stage (TU4), allowing the first stage to operate at increased gain. The signal-to RF noise ratio of the modified amplifier is therefore 24dB better than the original— a really significant improvement.
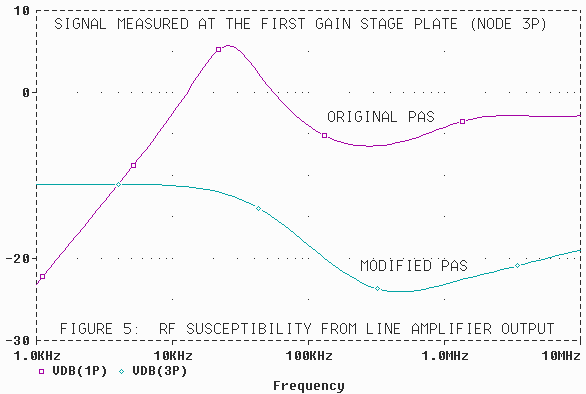
Fig. 5. RF susceptibility from line amplifier output (signal amplitude at the first gain stage plate with an input signal injected at the preamp output).
The Parts Connection Catalog & Resource Guide (1-800-769-0747; https://www.sonicfrontiers.com/tpc) contains an excellent collection of schematic diagrams of classic tube preamplifiers and power amplifiers. Nearly every amplifier with feedback— and that’s almost all of them— uses the traditional compensation technique with the capacitor in parallel with the feedback resistor. In all fairness, this technique is easy to apply and provides good stability with minimum frequency response loss. The new technique, Miller compensation in the input stage, requires two capacitors (C3M and C3C in fig. 2) to achieve good frequency response extension. With the proper component values, difficult to obtain without SPICE, the new technique has improved stability, i.e., less of a response peak with capacitance in shunt with the load. Compensating a feedback loop at the input stage also reduces response peaks at intermediate gain stages that can occasionally cause such problems as unexpected saturation.
Feedback in power amplifiers.
When an amplifier is driven to its limits— into saturation— the tops and bottoms of the output waveforms flatten out. This phenomenon is known as clipping. Because most music signals contain short-term peaks whose instantaneous power is much greater than the average, most amplifiers— even moderately powerful amplifiers— occasionally clip during normal operation. For this reason, the behavior of an amplifier during clipping has a profound effect on its sound quality. The power amplifier of fig. 6 is an excellent platform for studying the effects of feedback on clipping. It can be built as a modified Dynaco Mark 3, or from scratch with a 4300 ohm primary impedance output transformer. Due to the limitations of the evaluation version of PSpice, simplified bias and power supplies have been shown. I have named this amplifier the “Local Hero” because of its use of local feedback (and also after one of my favorite movies.). Since it has outstanding sound quality and makes exemplary use of feedback, we shall describe its circuit in some detail.
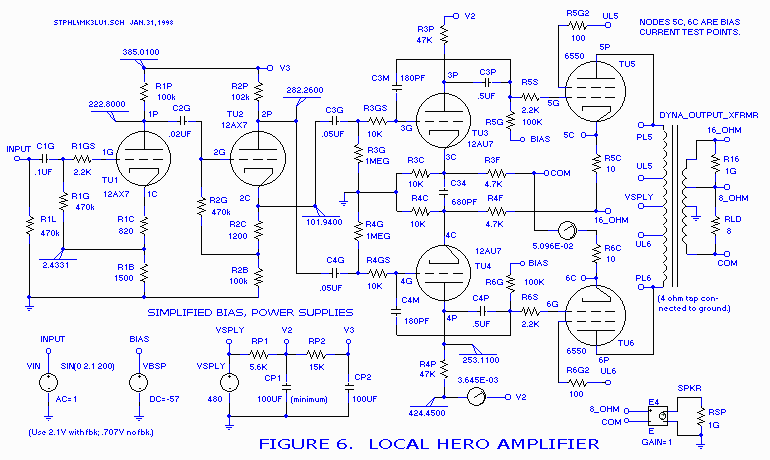
Figure 6. The “Local Hero” amplifier: a modified Dynaco Mark III
The Local Hero’s chief feature is the absence of global negative feedback from the output transformer secondary to the input stage. Instead, balanced feedback from the output is returned to the cathodes of the driver (TU3, TU4) and output tubes (TU5, TU6). This eliminates many of the stability problems associated with a large global loop: The fewer stages in the loop, the easier it is to control phase shifts at both frequency extremes. The amount of feedback on the drivers is controlled by voltage dividers (R3F, R3C) and (R4F, R4C). The resistance ratios may be changed to alter the amount of feedback, but if they are, the parallel resistance between the driver cathodes (nodes 3C and 4C) and ground should remain around 3300 ohms. The loop is compensated with capacitors C3M and C4M, with C34 added to extend the high frequency response. (These capacitors have the same functions as C3M and C3C in fig. 2.) They do not transmit RF interference from the output. The effects of RF interference are further reduced because the signal at the drivers is much greater than at the input. RLD and R16 are for simulation only– they aren’t physically present. RLD represents the speaker load. R16 prevents an unconnected node error. Stability is excellent: Even a 1 microfarad capacitor in shunt with the load results in little ringing.
The input stage (TU1) uses local current feedback: Gain can be controlled by adjusting R1B. It is ac-coupled to a split-load phase invertor (TU2), which also has local current feedback. The split-load phase invertor is an excellent performer when it doesn’t have to drive output tubes, as it does in the classic Dynaco circuits. Ac-coupling allows the plate resistors to be chosen for equal but opposite ac current in TU1 and TU2, so that no net ac current is drawn from the power supply. This reduces sonic degradation from power supply electrolytic capacitors, which are notoriously nonlinear. The original Williamson circuit (the grandfather of the Local Hero) had to be dc-coupled because there were already too many coupling capacitors in the global feedback loop; another would have undermined the low frequency stability. Ac-coupling also increases the phase invertor’s maximum output. A note for experimenters: If you build this circuit, you may need to make fine adjustments on C3M and C4M, and possibly also C34, for flat response and good stability.
Note: I haven’t actually built the Local Hero, but I’ve gotten inquiries. I haven’t included the power supply design. Power supplies for this type of amplifier are pretty standard. The Hammond 372JX (300-0-300V, 250 mA) and 373BX (350-0-350V, 175 mA) are good power transformer choices if you’re building Local Hero as monoblocks. The 372JX is more conservative, but the 373BX will should put out about 30% more power (you’ll need 500V power supply capacitors). If you build it, I recommend testing it by driving it with a square wave generator, loading it with a typical loudspeaker load (with cables), and looking at the signal at the output (and speakers) with an oscilloscope. If there is ringing, increase the Miller capacitors, C3M and C4M. If the square waves seem excessively rounded, you may be able to decrease C3M and C4M with no ill effects. The voltage at test points 5C and 6C should be approximately 0.5 V for 50 mA bias current (per tube). Remember to be careful of high voltages when you work inside tube amplifiers!
A pleasant surprise! Jorn Loe of Norway built a Local Hero. It took him a long time (he isn’t experienced at building tube amplifiers), but it’s working and sounding fine. You can reach him at n (dot) craft (at) c2i (dot) net.
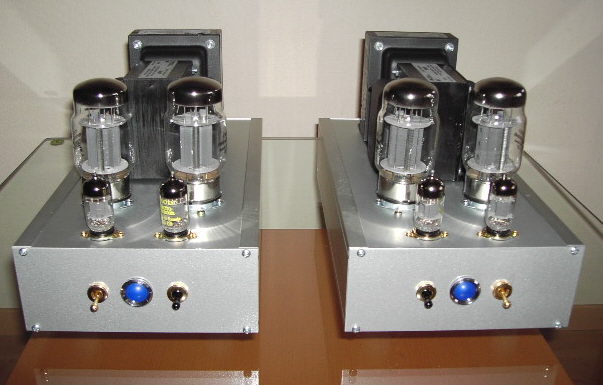
A comment from Jorn, Oct. 8, 2006: I did a small correction in the Local Hero after oscilloscope measurements showed some oscillation. My layout made the C3M and C4M paths too long. When I connected C3M and C4M directly to anodes 3P and 4P the amplifiers went silent. I talked to other tube builders, and they said that this was a good change. (Sounds fine to me. —NLK)
The results of driving the amplifier into saturation are shown in figures 7 (without feedback) and 8 (with feedback). Since the gain with an 8 ohm load is 9.46dB lower (a factor of 0.3365) with feedback than without it, the input signals have been adjusted for the same degree of saturation. The actual amount of feedback is the difference in gain without the load: 15.4dB. This is a moderate amount: much more than most single-ended tube amplifiers, slightly less than classic push-pull tube amplifiers, and much less than mid-fi solid-state amplifiers.
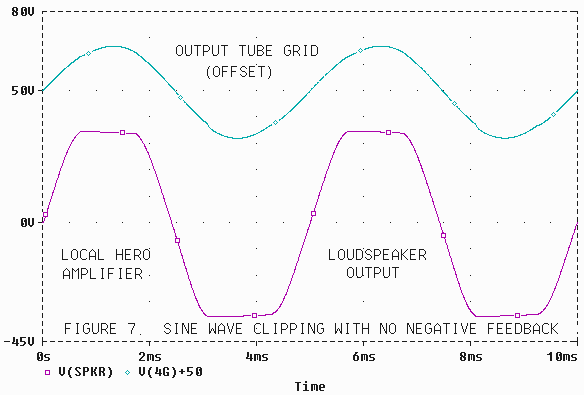
Fig. 7. Sine wave clipping with no negative feedback
When feedback is applied, clipping at the amplifier output ( lower curve, fig. 8) becomes more severe: its onset becomes more abrupt and the tops and bottoms of the waveforms become flatter. Since no signal is fed back during clipping, voltage spikes appear at the grid of the output tubes (upper curve, fig. 8). The difference between figures 7 and 8 is modest because only 15.4 dB of feedback has been employed: Had more been applied to emphasize the effects of clipping, the sound quality would have suffered, and the Local Hero would not have been such a good example of proper use of feedback. In a mid-fi solid-state amplifier, with 40 dB or more feedback, the tops and bottoms would have been cleanly chopped off.
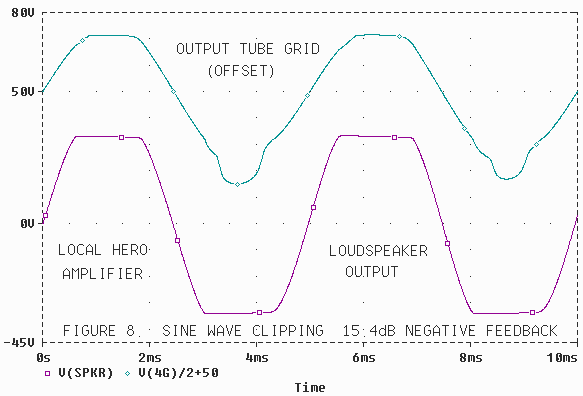
Fig. 8. Sine wave clipping with 15.4 dB negative feedback
Hard clipping is the direct cause of the constricted dynamics and much of the sonic harshness attributed to feedback. The audible degradation can only be determined by ear: there is no hard and fixed rule, but much can be learned by correlating SPICE results with careful listening. Sonic degradation is a strong function of amplifier power and loudspeaker efficiency: the higher the sound pressure level at the onset of clipping, the less offensive it will be. That, along with the stability/frequency response issue described above, is the reason that low powered SE amplifiers have limited tolerance for feedback.
Clipping is rarely a problem in preamplifiers. The figure 2 preamplifier has enough headroom to drive any commercial power amplifier well beyond saturation— 10 to 30 dB, depending on its sensitivity and input impedance.
Despite its drawbacks in power amplifiers, negative feedback offers one significant advantage: It reduces the output impedance. High output impedance can cause irregular frequency response under realistic loudspeaker loads [8] as well as poor woofer damping, audible as mushy ill-defined bass. The optimum output impedance and feedback level depends on a number of factors that ultimately reduce to a tradeoff between mushy bass at one extreme (no feedback) and harsh clipping at the other (too much feedback). Several aspects of this tradeoff are discussed in the sidebar.
Despite negative feedback’s disadvantages in single-ended tube power amplifiers, two local experimenters have chosen to use a small amount of it— around 3 to 4dB. Without it, the bass was just too sloppy. They did not take this step lightly: They listened extensively, perhaps obsessively, before adding feedback, and they wouldn’t have done so had they duplicated Martin Colloms’ observation, made on the Cary 805C, that even the smallest amount of feedback compromises musicality.
I can only speculate on the reasons for this discrepancy. At first I thought the sound pressure level at the onset of clipping would be slightly higher for the experimenter’s systems, which use Svetlana SV811-10 output tubes operated in class A2, driving loudspeakers with 93 and 96dB efficiency in rather small rooms. But the clipping level of the Cary 805C driving the 93 dB efficient Wilson WITT should be at least as high. Perhaps the discrepancy can be explained by differences in the room size and acoustics, amplifier clipping signature, or loudspeaker damping requirements. But there is another intriguing possibility.
Since I don’t have access to the Cary 805C’s schematic diagram, I can only speculate that when feedback is switched on, the traditional compensation technique— a capacitor in shunt with the feedback resistor— may be employed. If this is so, then all the RF picked up from the speaker cables will be transmitted back to the input stage, even for the smallest amount of feedback, but none will be transmitted if there is no feedback. This could well explain Martin Colloms’ observation.
Any circuit technique that improves an amplifier’s linearity at power levels below saturation, i.e., reduces total harmonic distortion (THD), will result in more abrupt, harder clipping. Negative feedback is only one of several such techniques. The relationship between linearity and clipping can be examined with the transfer and linearity curves in figures 9 and 10. These curves are derived from the same PSpice simulations as figures 7 and 8 for saturated 200Hz sine waves, but instead of using time as the independent variable (x-axis), they use the input voltage, V(INPUT).
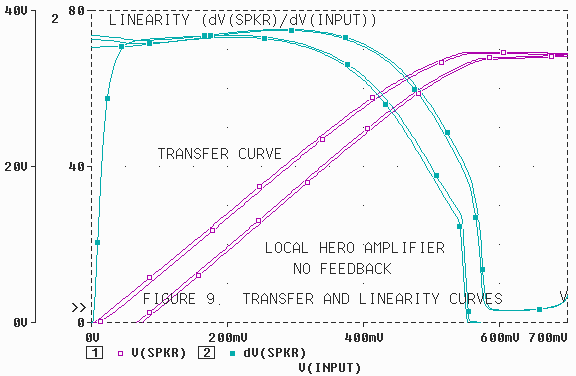
Figure 9. Transfer and linearity curves for the “Local Hero” amplifier without feedback
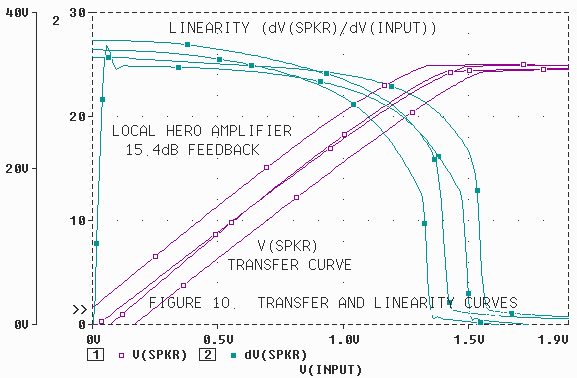
Fig. 10. Transfer and linearity curves with 15.4dB negative feedback
The transfer curve is simply the output voltage, V(SPKR), as a function of the input voltage. For small input signals, it rises along a relatively straight line. As it approaches saturation, it deviates from the straight line to a greater or lesser degree, depending on how well the circuit is linearized. At saturation, it flattens out. Since it can be difficult to see the deviation from the straight line, we introduce the linearity curve.
The linearity curve is the slope, or rate of change, of the transfer curve. In the language of differential calculus, it is the derivative of the output voltage with respect to the input, dV(SPKR)/dV(INPUT). For small input signals, it is a relatively straight horizontal line whose value equals the gain of the amplifier. As it approaches saturation, it starts to drop. This drop is much more visible than the corresponding change in the slope of the transfer curve. At saturation, it drops to zero.
We must now explain the multiple traces in the transfer and linearity curves. To obtain these curves, we drive the output tubes (via computer simulation, of course) into hard saturation— hard enough to drive the voltage on the grid of output tube positive with respect to the cathode for a portion of the cycle. This causes grid current to be drawn, alternately charging coupling capacitors C3P and C4P. These capacitors can charge quite rapidly, but their discharge is governed by the RC time constant that includes the grid resistor: C4P�R6G or C3P�R5G = 0.05 seconds, corresponding to a -3dB frequency of 3.2Hz. Since the simulation starts with no signal, and hence no charge, the charge that accumulates whenever one of the output tube grids conducts causes the multiple traces.
This is no mere artifact of SPICE simulation: It is a real effect in any amplifier where the grids of the output tubes are capacitively coupled. In hard saturation, continuous waveforms behave very differently from a single transient pulse: They can build enough charge across the coupling capacitors to drive the output tubes deep into cutoff. This leads to an overload recovery time, governed by the above-mentioned RC time constant, that can be quite audible. Overload recovery is eliminated in class A2 or AB2 amplifiers, where the output tube drivers can source grid current. This can be accomplished either with transformer-coupling or dc-coupled cathode followers [7], either of which are costly. An additional advantage of class A2 or AB2 power amplifiers is that they can put out much more power when the output stage is triode-connected.
Now, back to the transfer and linearity curves. Fourier analysis tells us that signals on straight line portions of the curves have no appreciable distortion. Signals on portions of the curves with sharp kinks or abrupt changes generate lots of high order harmonic distortion. Depending on your viewpoint, either the kinks or the high-order harmonics are the source of sonic degradation. Signals on portions of the curves with gradual curvature or gentle rounding generate mostly low (second and third) order harmonic distortion.
In a circuit with high linearity— the result of large amounts of negative feedback— the transfer curve is a straight line up to saturation, where is abruptly levels off. The linearity curve is a horizontal line up to saturation, where it “hits a brick wall” and abruptly drops to zero. There will be very little harmonic distortion below saturation, but as soon as the amplifier saturates, serious high-order harmonic distortion is generated.
Amplifiers are traditionally specified for total harmonic distortion at the rated power, typically around 1dB below saturation (roughly 90% of saturation voltage or 80% of saturation power). The difference between the rated power and the saturation level is the “headroom.” An amplifier with relatively soft clipping, such as the Local Hero without feedback (fig. 9), must start rounding off the signal at amplitudes well below saturation. At the rated power therefore, it can have considerable THD, but this distortion is mostly low order (second and third) harmonics. At power levels above saturation, high order harmonic distortion components will remain much lower than for a highly linear amplifier.
The problem of hard clipping is not limited to feedback amplifiers. I once had an amplifier— obtained in a trade, without careful auditioning— that featured a circuit called “super-feedforward,” intended to eliminate the vices of feedback. It put out 120 watts per channel at 0.005% THD (one of the lowest in the 1980 Audio Annual Equipment Directory), had response to 250kHz, and had sound that can only be described as hateful and irritating, even though it had no obvious flaws. The only suspicious measurement was the saturated sine waves: They didn’t just clip; they dipped! I didn’t keep the amplifier long enough to find out why.
Modest levels of low order harmonic distortion do not seem to harm sound quality. In SE tube amplifiers, most of which have little or no feedback, the predominant distortion component is the second harmonic. Push-pull amplifiers cancel even order harmonic distortion components, resulting in lower THD. The second harmonic distortion in SE amplifiers may well be responsible for their alluring sound. It has given rise to the theory that even order harmonic distortion components are more benign than odd. My own view is that the real culprit is high order distortion components. Second harmonic distortion may actually enhance sound quality by adding harmonic content— some call it “euphonic distortion”— that increases the perception of richness and detail. In all fairness, I haven’t persuaded my experimenter friends that this is the case.
In the 1950s and early 1960s, high fidelity tube amplifiers had 0.5 to 1.5% THD at rated power output. In the 1970s, a distortion race took place that paralleled the automotive horsepower race of the 1950s: If 1% THD was OK, 0.1% was better, and 0.001% would be really awesome. The more leading zeros, the merrier. These low THD levels were primarily accomplished by increasing negative feedback to levels well beyond 40dB. We all know the results: amplifiers that sounded so bad that— a real silver lining on a dark cloud — they gave birth to the high-end audio industry we know today.
That brings us to the ultimate audio paradox: In a well-designed high fidelity power amplifier, the lower the total harmonic distortion at rated power, the harder the clipping; hence the worse the sound. This runs exactly counter to the popular belief of the past, a belief still widely held in segments of the audio industry outside the high-end. Within the high-end community, the predominant belief has been that THD has little to do with sound quality, even though most of us have experienced evidence of the inverse correlation: mid-fi amplifiers with low THD but mediocre sound and old tube amplifiers much higher THD but wonderful sound.
To minimize the likelihood of misunderstanding— inevitable when the above statement is quoted out of context— let me emphasize that the power amplifier must have no serious design flaws (like low-level crossover distortion) and must be working properly. Extremely high levels of THD, over 5 or 10%, probably indicate trouble. Low harmonic distortion is still desirable in preamplifiers and line level amplifiers that have at least 10dB of headroom (a factor of ten in power) between their rated output and saturation. Low harmonic distortion at rated output only degrades the sound quality of power amplifiers.
The one conventional measurement that provides some indication of an amplifier’s saturation behavior— THD+noise as a function of power level— has several shortcomings. It emphasizes harmonic distortion at levels below saturation and lacks sufficient detail above saturation to describe actual clipping. Furthermore, it measures total harmonic distortion; hence it gives far too much weight to the relatively benign second and third order distortion components. The transfer and linearity curves (figures 9 and 10) are more direct indicators of audio quality and saturation behavior. The transfer curve is easy to obtain on any oscilloscope with X-Y input capability. The linearity curve is trickier because it involves differentiating the output voltage with respect to the input. This requires a digital oscilloscope. A number of issues need to be resolved before this measurement becomes a standard: How far should the amplifier be overdriven? What is the ideal waveform for this measurement? We chose a 200Hz sine wave because, with a 1kHz sine wave, the amplifier’s high frequency limitations smoothed the curves. Would a 100Hz sine wave or a single sawtooth pulse have been any better? It would be worthwhile to publish these curves in equipment reviews, even before a standard is established, to see what can be learned.
Measurements made inside the circuit, examples of which are found in figures 3-5, have great value for the equipment designer, but little value for the reviewer because different amplifiers have such different circuit topologies. There is a real need for measurements that indicate sensitivity to RF interference. Paul Miller made a good first step by inserting noise-modulated RF signals into amplifiers and measuring the audible noise spectrum [5], but his descriptions were incomplete, and I am unaware of any follow-up work.
To feedback’s opponents, a well-designed amplifier without feedback is preferable a similar amplifier with it. Presumably, this also includes individual devices like tubes or transistors. There is an important, though not obvious, exception that casts doubt on this view. The triode— the favorite of audiophiles— has built-in local negative feedback. In a plate-follower triode gain stage, as the grid voltage (the input signal) increases, the plate current increases. This causes the plate voltage (the output signal) to drop. Since the plate current depends on the plate voltage, it will be lower than it would have been had the plate voltage remained constant. This, by definition, is degenerative, i.e., negative feedback: It could be eliminated by making the plate current independent of the plate voltage. This could be done inside the tube by adding a second grid, connected to a constant high voltage, between the control grid and plate. To keep the electrons that bounce off the plate and getting absorbed by the second grid, a third grid, connected to a low voltage, would have to be added between the second grid and the plate.
What would such a tube be called? A pentode, of course! With no negative feedback, its gain and output impedance are much higher and its linearity is poorer. The sound of the triode is almost universally preferred. Considered in this light, feedback shouldn’t look so bad. For a tube amplifier to be called truly feedback-free— in the local as well as the global sense— it would have to be built entirely of pentodes. Any takers?
By incorporating engineering theory into computer simulation, we have been able learn a great deal— some of it new and unexpected— about the origins of audio quality. The old theory was fine as far as it went; it just couldn’t handle the complexity of audio amplifiers, where the devil is truly in the details. The use of computer simulation to study subjective sound quality is still in its infancy. As it progresses, amplifier designs will become simpler, more elegant, and better sounding. Best of all, amateurs are now in a position to make real contributions to the art, much as they did in the early days of radio. Some excellent simulation tools are available for free, including the evaluation version of PSpice from MicroSim, and Duncan Munro’s excellent web page, https://duncanamps.simplenet.com/. Relevant articles appear in Glass Audio and Audio Electronics.
Extensive computer simulation and listening have led me to conclude that feedback amplifiers can have flawless sound quality, without a trace of muddiness or grayness, provided that the three dangers of feedback— instability, RF susceptibility, and sharpened clipping— can be eliminated or minimized. This can be always be accomplished in well-designed vacuum tube preamplifiers, but there will always be a tradeoff between clipping and output impedance in power amplifiers. Because this tradeoff involves many factors— amplifier saturation level, open loop output impedance, loudspeaker efficiency and damping, etc. — one solution will never fit all needs. The industry-wide trend towards lower negative feedback may well continue, but I will hazard the guess that it won’t go all the way to zero.
Although I cannot prove that feedback has no audible drawbacks— no such proof is possible when the issue is subjective quality— I can offer the fig. 2 preamplifier line stage as evidence. When implemented with a high quality power supply, its superb sound should equal anything on the market, with or without feedback. Details of its construction (as a modified Dynaco PAS) have been published in Glass Audio [4]. The entire project, which includes a moving magnet phono stage, can be built for around $300 in parts.
In high fidelity amplifiers, the magic is in the circuit. Premium components and skilled layout may improve the sound of a good circuit, but they cannot save a poor one. With the knowledge gained by correlating SPICE simulations with careful listening, we can confidently apply the right amounts feedback to the right places— sparingly in power amplifiers, more generously in preamplifiers— without fear of degrading the sound. Advertising claims of “no negative feedback” should be treated with the usual skepticism. A well-designed amplifier can have excellent sound whether or not it has feedback. The ultimate judge of audio quality is, and always will be, the ear.
In a power amplifier with pentode output tubes (6L6GC, EL34, 6550, etc.), output impedance and power depend on the screen grid connection. The three standard connections are:
The following table compares conventional expectations with PSpice simulations for the Local Hero amplifier, changing only the output tube screen grid connections.
Power output is measured at clipping for 50mA bias current per tube and a 480V power supply. Normal rated power output is about 20% lower. The UL connection is popular in classic designs because it offers a good tradeoff between output power and impedance. Changing an amplifier from UL to triode mode is a common modification. Triode mode has lower output impedance, less sensitivity to loudspeaker loads, and less feedback (hence softer clipping) if the circuit is unchanged, but also lower power. If the Local Hero is wired in triode mode, reduce C3M and C4M to 150pF. A class A2 or AB2 triode output stage [7], which can operate with grid current, would have much greater output power. It’s the best of both worlds, but it comes at a price: The output stage grid must be driven by a transformer-coupled gain stage (limited bandwidth and don’t even think of feedback) or by direct-coupled cathode followers, which greatly complicates the power supply. This has been accomplished in The Emperor’s New Amplifier. |
- Scott Reynolds, “Vacuum-tube models for PSPICE simulations,” Glass Audio, vol. 5, no. 4, 4/93 p. 17.
- W. Marshall Leach, Jr., “SPICE models for vacuum-tube amplifiers,” J. Audio Eng. Soc. Vol 43, No. 3, March 1995, p. 117.
- Norman L. Koren, “Improved Vacuum-Tube Models for SPICE Simulations,” Glass Audio, Vol. 8, No. 5, 1996, p. 18.
- Norman L. Koren, “SPICE and the Art of Preamplifier Design,” (in two parts), Glass Audio, Vol. 8, No. 2, p. 1 and No. 4, p. 38, 1997.
- Paul Miller, “Resonances and Repercussions,” Hi Fi News & Record Review, June 1989, p. 35.
- Paul W. Tuinenga, “SPICE, A Guide to Circuit Simulation & Analysis using PSpice,” Prentice-Hall, 1992 or 1995.
- Scott Frankland, “The Magic of Design and Synergy, Part I: The Wavestream V-8 Triode,” Positive Feedback, Vol. 5, No. 5, 1995, p. 23.
- John Atkinson, “Real-life measurements,” Stereophile, August 1995, p. 168.
About the author Norman Koren, a native of Rochester, NY, received a BA in physics from Brown University in 1965 and an MA in physics from Wayne State University in 1969. His destiny as a high-tech nomad has taken him to Boston, Philadelphia, Silicon Valley, San Diego, and most recently to Colorado, where he worked in research and development of digital magnetic recording channels through 2001.
This page has been reproduced with permission of Norman Koren.
January 2016